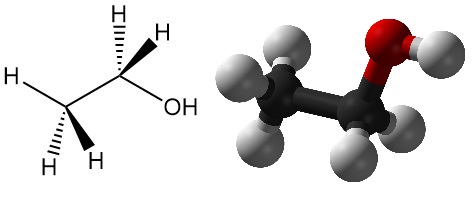
What is Ethanol?
Ethanol, also known as ethyl alcohol, is one of the most important alcohols, with the chemical formula CH3CH2OH. It is a colorless, volatile liquid with a characteristic odor and is widely used in a diverse range of applications. Other names of ethanol include alcohol spirit, spirit of wine, grain alcohol, absolute alcohol, and ethyl hydrate.
The term “alcohol” originates from the Arabic words “al” and “kohl,” referring to a finely ground antimony powder used for cosmetic purposes. Over time, the meaning evolved to denote a high degree of fineness or purity, eventually becoming specific for ethanol, “spirits of wine rectified to the highest degree.”
Ethyl alcohol is a well-known component of alcoholic beverages, which are produced through the fermentation of fermentable carbohydrates. The fermentation broth itself may be consumed as a beverage, as in the case of beer or wine, or the alcohol can be concentrated to produce spirits with higher alcohol content.
Beer normally has 4–6% by volume of ethanol, wine contains 12–14%, and spirits can range from 30–50% by volume. Other alcoholic beverages have different quantities of ethanol.
For industrial and commercial uses other than alcoholic beverages, ethanol is denatured by adding substances like methanol, pyridine, formaldehyde, or sublimate. Denatured ethanol is used as a solvent, raw material for chemical manufacturing, and fuel.
The history of ethanol traces back to ancient times. Fermented beer was consumed in Babylon, while wine production dates back to around 3000 BC in ancient Egypt, where it was prepared and consumed by the pharaohs.
The distillation process likely originated between the 10th and 14th centuries. During this time, the “spiritual” effects of ethanol were recognized, leading to the term “spiritus” for alcoholic drinks. Early wine distillates were primarily used for medicinal purposes.
Until the 17th century, alcoholic fermentation was considered a spoilage process. The nature of fermentation was initially clarified in the 19th century with the discovery of the microscope, which revealed yeast cells as living organisms. However, it took approximately 150 years to recognize the role of these organisms in the fermentation process.
Table of Contents
1. Physical Properties of Ethanol
Pure ethanol (absolute alcohol) is a volatile, flammable, clear, and colorless liquid with a pleasant odor. It is miscible with water, ether, acetone, benzene, and some other organic solvents. Anhydrous ethanol is hygroscopic.
Ethanol forms an azeotropic mixture with water, which contains 95.57 wt % ethanol and 4.43 wt % water. Therefore, the highest concentration of ethanol that can be obtained by distillation of an ethanol-water mixture is 95.57 wt%. Azeotropic distillation, with the help of a tertiary solvent (e.g., benzene), must be introduced to produce absolute (anhydrous) ethanol.
The important physical properties of anhydrous ethanol are listed in the following table:
Property | Value |
---|---|
CAS Number | [64-17-5] |
Chemical Formula | C2H5OH |
Molecular Weight | 46.7 g/mol |
Freezing Point | -114.1 °C |
Boiling Point | 78.32 °C |
Critical Temperature | 243.1 °C |
Critical Pressure | 6383.48 kPa |
Critical Volume | 0.167 L/mol |
Density | 0.7893 g/mL |
Refractive Index | 1.36143 |
Surface Tension (at 25 °C) | 23.1 mN/m |
Viscosity (at 20 °C) | 1.17 mPa·s |
Solubility in Water (at 20 °C) | Miscible |
Heat of Vaporization (at normal boiling point) | 839.31 J/g |
Heat of Combustion (at 25 °C) | 29676.69 J/g |
Heat of Fusion | 104.6 J/g |
Flammable Limits in Air | Lower: 4.3 vol % Upper: 19.0 vol % |
Autoignition Temperature | 423 °C |
Flash Point | 14 °C (closed-cup) |
Specific Heat (at 20 °C) | 2.42 J/(g·°C) |
Thermal Conductivity (at 20 °C) | 0.170 W/(m·K) |
Dipole Moment (liquid at 25 °C) | 5.67 × 10-30 |
Magnetic Susceptibility (at 20 °C) | 0.734 × 10-6 |
Dielectric Constant (at 20 °C) | 25.7 |
2. Chemical Reactions of Ethanol
The chemical reactivity of ethanol is primarily influenced by its hydroxyl (-OH) group, which participates in numerous industrially significant reactions such as dehydration, halogenation, ester formation, and oxidation.
The hydrogen atom of the hydroxyl group can be replaced with an active metal, such as sodium, potassium, or calcium, to form metal ethoxides (ethylates) with the evolution of hydrogen gas.
CH3CH2OH + M → CH3CH2OM + 1/2 H2
Sodium ethoxide, a commonly used metal ethoxide, can be prepared by the reaction of absolute ethyl alcohol with sodium or by refluxing absolute ethyl alcohol with anhydrous sodium hydroxide. Commercially, water is removed from the reaction mixture using azeotropic distillation with benzene.

Sodium ethoxide readily hydrolyzes to yield ethyl alcohol, sodium ions, and hydroxide ions.
Sodium ethoxide is used as a condensing and reducing agent in organic synthesis. It reacts with sulfur monochloride to produce diethyl thiosulfite. Additionally, sodium ethoxide is a reagent for the commercial production of barbiturates, ethyl orthoformate, and other chemicals.
Aluminum and magnesium can also react with ethanol to form ethoxides, but this reaction requires catalysis by amalgamating the metal.
Well-cleaned aluminum filings react with ethanol at room temperature in the presence of mercuric chloride. This reaction can be carried out in an autoclave at elevated temperatures without a catalyst or with the addition of sodium ethoxide.
The hydroxyl group of ethanol can also participate in other reactions, such as the ring-opening of epoxides to form hydroxy ethers and the addition to acetylene to form ethyl vinyl ether. These reactions are catalyzed by both acidic and basic conditions.
The acid-catalyzed addition of ethanol to acetylene or to vinyl ether produces acetals, which are also produced by the acid-catalyzed reaction of ethyl alcohol with an aldehyde or ketone.

The hydroxyl group can be replaced by halogens from inorganic acid halides or phosphorus halides, leading to the formation of ethyl esters of the acid and an ethyl halide. Phosphorus trichloride and thionyl chloride are commonly used to produce ethyl chloride, with triethyl phosphite and diethyl sulfite as byproducts.

Halogen acids can also react with ethanol to produce alkyl halides. The reactivity of the halogen acids varies, with hydroiodic acid being the most reactive. Ethyl chloride can be prepared by the reaction between ethanol and hydrogen chloride.
CH3CH2OH + HX → CH3CH2X + H2O
The reaction of ethanol with hydrogen bromide yields ethyl bromide, which is a precursor for the ethyl Grignard reagent and various ethyl amines.
2.1. Esterification
Ethanol reacts with inorganic and organic acids, acid anhydrides, and acid halides to produce esters. If an inorganic acid containing oxygen, such as sulfuric acid or nitric acid, is reacted with ethyl alcohol, the resulting ester contains a carbon-oxygen bond that can be hydrolyzed.
Organic esters are formed by a dehydration reaction between ethanol and an organic acid. This reaction is reversible and reaches equilibrium slowly. Acidic catalysts such as sulfuric acid, hydrochloric acid, boron trifluoride, and p-toluenesulfonic acid are commonly employed to accelerate the esterification process.
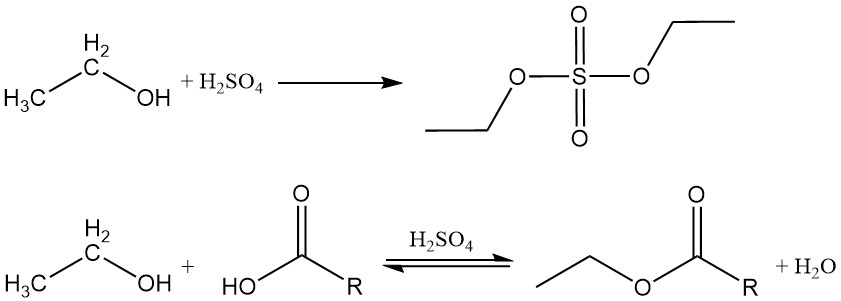
Ethanol also reacts with acid anhydrides or acid halides to form the corresponding esters.
The direct conversion of ethanol to ethyl acetate is believed to proceed through acetaldehyde and its subsequent condensation (Tishchenko reaction). An ethyl acetate yield of approximately 24% can be achieved using a copper oxide catalyst containing 0.1-0.2% thoria at 350 °C.
2.2. Dehydration
Ethanol can undergo dehydration to produce ethylene or ethyl ether. The specific product formed depends on the reaction conditions.
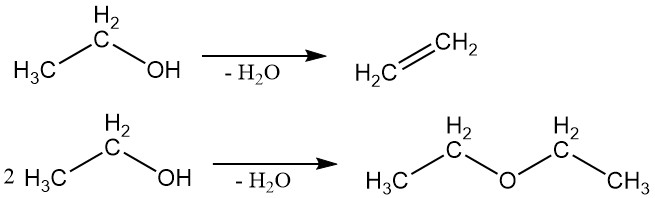
2.3. Dehydrogenation
Ethyl alcohol can be dehydrogenated to acetaldehyde in a vapor-phase reaction using suitable catalysts.
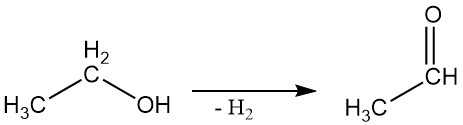
2.4. Haloform Reaction
Ethyl alcohol reacts with sodium hypochlorite to yield chloroform, a reaction known as the haloform reaction.
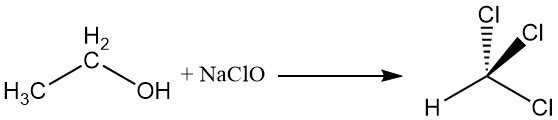
3. Industrial Production of Ethanol
Ethanol is produced by both synthetic and fermentation processes. One of the earliest synthetic routes involved the reaction of ethylene with sulfuric acid, a process discovered over a century ago.
However, the industrial-scale production of synthetic ethanol did not become economically feasible until the 1930s, when the Carbide and Chemical Corporation developed the indirect hydration process.
This process, which involved the absorption of ethylene in sulfuric acid followed by hydrolysis, has since been largely replaced by the direct catalytic hydration of ethylene, introduced by Shell Chemical Company in 1948.
In recent decades, alternative methods for ethanol synthesis have emerged, using coal-based starting materials such as methanol or synthesis gas. These processes include homologation, carbonylation, and the direct conversion of synthesis gas to ethanol.
However, fermentation of sugar, starch, or cellulose remains the predominant method for ethanol production, accounting for up to 90% of global output.
3.1. Production of Ethanol by Direct Catalytic Hydration of Ethylene
The hydration of ethylene to ethanol is a reversible reaction. The equilibrium constant for this reaction is influenced by temperature and pressure. Higher temperatures favor higher ethylene conversion but also increase the rate of side reactions, such as the formation of diethyl ether.
Kinetic studies have been conducted on various catalysts, including phosphoric acid-silica gel and blue tungsten oxide-silica gel. These studies have provided insights into the activation energy and reaction rate under different conditions.
Several process variables, including pressure, temperature, and the water-to-ethylene molar ratio, impact the ethylene conversion. Under typical reactor conditions (approximately equimolar ethylene-water feed, 250–300 °C, 5–8 MPa), the equilibrium conversion of ethylene ranges from 7 to 22%.
In addition to the desired hydration reaction, side reactions can occur, leading to the formation of byproducts. At lower temperatures, diethyl ether can be produced, while at higher pressures, polymerization of ethylene to butenes and higher olefins may take place.
The proposed mechanism for ethylene hydration involves four steps:
- A proton is added to the ethylene molecule to form a π-complex.
- The π-complex is converted to a carbocation.
- A water molecule is added to the carbocation.
- A proton is removed from the protonated ethanol to yield ethanol.
Catalysts
The hydration of ethylene to ethanol is catalyzed generally by acidic catalysts. Phosphoric acid supported on various materials, including diatomaceous earth (kieselguhr, celite), montmorillonite, bentonite, silica gel, and opoka, is among the most widely used catalysts for this reaction.
In addition to phosphoric acid-based catalysts, other catalysts have been investigated, such as acidic oxides, heteropoly acids, zeolites, and salts. These materials offer potential advantages in terms of activity, selectivity, and stability.
Under reaction conditions, the support must be resistant to phosphoric acid but able to retain it in large amounts; it must also have adequate mechanical strength and a sufficiently long life; and, ultimately, it must provide an active, selective catalyst for the hydration.
To optimize catalyst performance, the support is pretreated with mineral acids, such as hydrochloric or sulfuric acid, to remove impurities like iron and aluminum, and impregnated with 50 to 77% phosphoric acid.
Heat treatment at elevated temperatures (700–1100 °C) is often used to enhance the binding of phosphoric acid to the support. While this treatment may slightly reduce the catalyst’s initial activity and selectivity, it can significantly improve its long-term stability and durability.
Production Process
The catalytic hydration of ethylene to ethanol involves a series of steps, as illustrated in Figure 1.
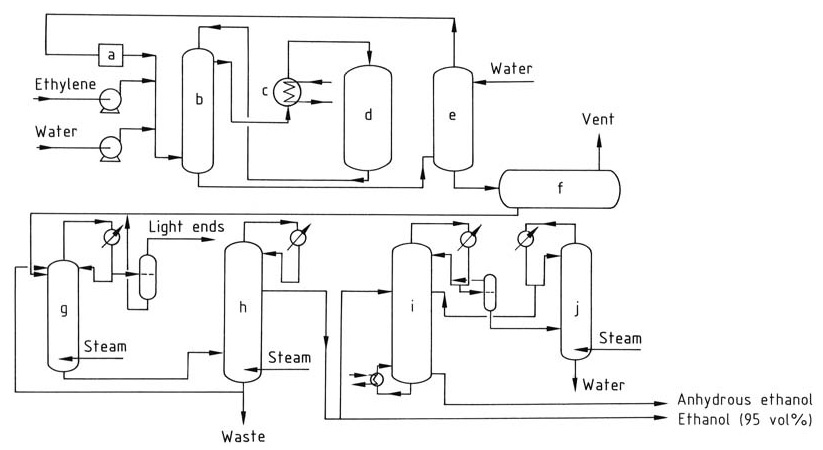
a) Circulation compressor; b) Heat exchanger; c) Superheater; d) Reactor; e) Washer; f) Crude ethanol tank; g) Extractive distillation column; h) Concentration column; i) Dehydration; j) Concentration column
Ethylene and deionized water, in a molar ratio ranging from 1:0.3 to 1:0.8, are heated to 250–300 °C at 6–8 MPa by passing through a heat exchanger and a superheater. The reaction is exothermic, releasing heat that can be recovered and used to preheat the feed stream.
The gas stream is washed with water to remove any residual ethanol. The purified gas is then recompressed and recycled back to the reactor to maintain the desired ethylene concentration.
The reaction products are cooled and condensed to separate the liquid and gaseous phases. The crude ethanol product collected in the washer sump contains approximately 10–25% ethanol by weight and is further purified by distillation to obtain a 95% ethanol-water azeotrope.
To produce anhydrous ethanol, the 95% ethanol can be dehydrated using azeotropic distillation.
During the catalytic hydration of ethylene, a portion of the phosphoric acid catalyst can be entrained by the gas stream. To prevent acid buildup in the downstream equipment, a dilute sodium hydroxide solution is injected to neutralize it.
The phosphoric acid lost due to entrainment and evaporation is replenished by periodically spraying it onto the catalyst bed to ensure a consistent catalyst concentration throughout the reaction process.
Diethyl ether, approximately 2%, is a common byproduct of the reaction and can be recovered and purified for separate use or recycled back to the reactor. Acetaldehyde, a potential impurity, is converted to ethanol by hydrogenation to avoid the formation of undesirable compounds like crotonaldehyde.
The process equipment consists of reactors, heat exchangers, distillation columns, and associated piping. Reactors are typically made of steel with a copper or carbon lining to protect against corrosion by phosphoric acid. Heat exchangers and piping exposed to phosphoric acid are also constructed of copper or copper alloys.
The process generates minimal waste. Vented ethylene, which accounts for less than 15% of the total feed, can be returned to the ethylene plant or incinerated. Wastewater from distillation is low in organic compounds and phosphate content (approximately 0.3 kg of Na2HPO4 per cubic meter) and can be easily disposed of.
3.2. Production of Ethanol by Indirect Hydration of Ethylene
The indirect hydration of ethylene using sulfuric acid involves two primary steps: the absorption of ethylene and the hydrolysis of the produced sulfate.
Ethylene reacts with concentrated sulfuric acid to form ethyl hydrogen sulfate and diethyl sulfate, which are then hydrolyzed with water to produce ethanol and diethyl ether.
Kinetic studies have shown that the absorption and hydrolysis steps follow a similar reaction mechanism as the direct hydration process but are influenced by the solubility of ethylene in sulfuric acid.
The final products of the indirect hydration process are ethanol and diethyl ether (5–10%). The yield of diethyl ether can be controlled by adjusting reaction conditions, such as the ratio of ethylene to sulfuric acid and the hydrolysis conditions.
The ethyl sulfates can be transesterified with acetic acid at 104 °C to produce ethyl acetate. Ethyl acetate is then recovered by distillation and hydrolyzed to ethanol and acetic acid. This process offers an advantage over the traditional hydrolysis method by avoiding the need for sulfuric acid reconcentration.
Production Process
Figure 2 shows the flow scheme for the production of ethanol by indirect hydration of ethylene.
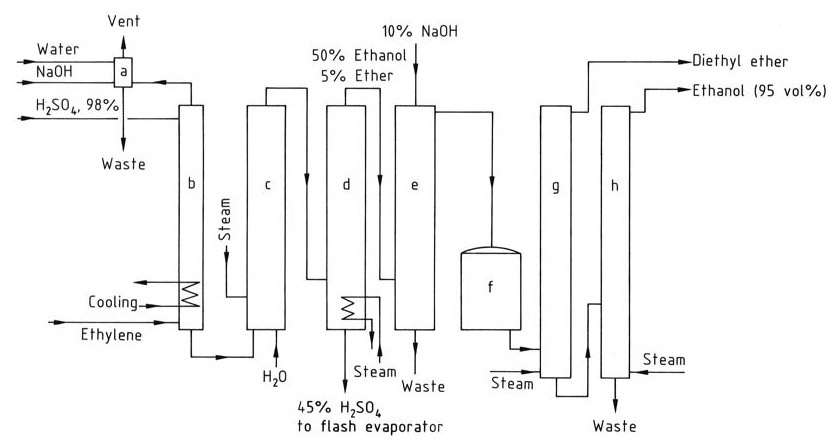
a) Wash column for residual gas; b) Absorber; c) Hydrolyzer; d) Stripping column; e) Wash column for crude ethanol; f) Crude product tank; g) Diethyl ether distillation column; h) Ethanol distillation column
The feed gas for the indirect hydration process must contain a minimum of 35% ethylene and should be free from higher homologues that can cause resin formation. Inert gases like methane and ethane can be added.
The absorption of ethylene increases with pressure, which is generally between 1 and 3.5 MPa. Each mole of sulfuric acid can absorb up to 1.4 moles of ethylene. The absorption is conducted in wash towers at 65–85 °C, and temperatures above 90 °C must be avoided to prevent resin formation.
The absorbed ethylene reacts with sulfuric acid to form ethyl sulfates after agitation for several hours. The hydrolysis of these sulfates is carried out in two stages: a low-temperature (70 °C) hydrolysis to convert diethyl sulfate and a subsequent high-temperature (100 °C) hydrolysis for about 1 hour to complete the reaction.
The sulfuric acid is diluted to 40–55 wt%, and ethanol and diethyl ether are recovered from the reaction mixture through a stripping column. The product mixture is then neutralized with sodium hydroxide, and the diethyl ether is removed in a separate distillation column.
The ethanol is further purified by distillation to obtain a 95% ethanol-water azeotrope.
Reconcentrating the dilute sulfuric acid is an expensive step of the indirect hydration process, characterized by high energy consumption, corrosion problems, and organic compound oxidation (the formation of tar).
To overcome these challenges, operating at lower pressures below 0.5 MPa and adding silver sulfate up to 7% to the sulfuric acid can improve the process efficiency and reduce reconcentration requirements.
The choice of construction materials depends on the specific process steps. Steel is commonly used for absorption and distillation equipment. Lead and acid-resistant materials are suitable for hydrolysis, while silumin, tantalum, cast iron, and lead are used for sulfuric acid reconcentration.
3.3. Other Methods
Other methods for synthesizing ethanol from methanol and synthesis gas have been developed. These processes are likely to be of industrial importance in the future because they use starting materials that are available from coal and not derived from petroleum.
3.3.1. Homologation of Methanol
Homologation, a process that extends the carbon chain of an alcohol by one carbon atom, has been explored as an alternative route for ethanol synthesis. Methanol can react with synthesis gas (CO + H2) in the presence of a cobalt carbonyl catalyst to produce ethanol.
Early attempts at methanol homologation resulted in low ethanol yields and complex product mixtures. However, advancements in catalyst design and reaction conditions have improved selectivity for ethanol.
Studies have shown that using tetrahydrofuran as a solvent or adding iodine or iodide with organic phosphines can enhance ethanol selectivity.
3.3.2. Carbonylation of Methanol and Methyl Acetate
Another approach to producing ethanol is the carbonylation of methanol to acetic acid, followed by hydrogenation. This method is based on the Monsanto process for acetic acid synthesis, which uses a rhodium carbonyl-iodine catalyst.
While direct hydrogenation of acetic acid to ethanol is possible, it requires high-pressure equipment and can be corrosive. A more practical method is the esterification of acetic acid with ethanol to form ethyl acetate, followed by hydrogenolysis of ethyl acetate to ethanol.
Several variations of the carbonylation process have been developed, including the use of methyl acetate as the starting material. Methyl acetate can be carbonylated to acetic anhydride, which is then reacted with ethanol and methanol to produce ethyl and methyl acetates. The ethyl acetate can subsequently be hydrogenolyzed to ethanol.
3.3.3. Conversion of Synthesis Gas to Ethanol
Synthesis by Heterogeneous Catalysis
Efforts to convert synthesis gas (CO + H2) to ethanol have focused on modifying existing catalysts used for methanol synthesis. Studies have shown that incorporating alkali metals, cobalt, or other elements can improve ethanol yields.
Research institutions like the Institut Français du Pétrole have developed complex catalysts containing multiple elements, including copper, cobalt, chromium, potassium, lanthanum, and others. These catalysts can produce alcohol mixtures containing a significant amount of ethanol, methanol, and higher alcohols.
Platinum-group metal catalysts, such as rhodium and lanthanum trioxide, have also shown promise in converting synthesis gas to ethanol. These catalysts can achieve high ethanol selectivities at 200–240 °C and atmospheric pressure conditions.
Synthesis by Homogeneous Catalysis
Homogeneous catalysis using ruthenium complexes has been explored for the conversion of synthesis gas to ethanol. Ruthenium catalysts, in combination with organic phosphorus compounds, can achieve ethanol selectivities of over 50%.
Various solvents and additives, such as tripropylphosphine oxide, tetrabutylphosphonium bromide, and alkyltriphenylphosphonium bromides, have been investigated to optimize ethanol production.
3.4. Production of Ethanol by Fermentation
Fermentation, a biosynthesis process, has been employed for centuries to produce various products, including ethanol. While corn has been the primary feedstock in recent decades, other agricultural materials, such as molasses, grains, sugar cane, beets, fruit, whey, and sulfite waste liquor, can also be used.
Research is ongoing to explore the feasibility of fermenting waste materials like garbage.
The suitability of different raw materials for ethanol production depends on factors such as availability, cost, sugar content, and ethanol yield. Some agricultural products, like potatoes, may be economically less attractive due to low ethanol yield and high transportation costs.
Fermentable materials can be broadly categorized into three types:
- Sugars: Direct fermentation of sugars obtained from sugarcane, sugar beets, molasses, or fruits is possible.
- Starches: Starches from grains, potatoes, or root crops require hydrolysis into fermentable sugars using enzymes from malt or molds.
- Cellulose: Cellulose-rich materials, including wood, agricultural residues, and sulfite waste liquor, must be converted to sugars using mineral acids before fermentation.
Fermentation is the conversion of sugars into ethanol and carbon dioxide under anaerobic conditions. While various microorganisms can ferment sugars, yeasts, particularly Saccharomyces cerevisiae, are commonly used due to their high ethanol tolerance and efficiency.
The fermentation of glucose proceeds through the glycolysis pathway, which is a series of enzymatic reactions that break down glucose into pyruvate, producing ATP (adenosine triphosphate) as energy. Under anaerobic conditions, pyruvate is converted to acetaldehyde, which is then reduced to ethanol by alcohol dehydrogenase.
Optimal fermentation conditions vary depending on the yeast strain, raw material, and desired product specifications. Key factors influencing fermentation are:
- Temperature: Most yeasts prefer a temperature range of 25–30 °C for optimal growth and ethanol production.
- pH: The pH should be maintained within a specific range, typically between 4.5 and 5.5, to ensure optimal yeast activity and prevent bacterial contamination.
- Nutrient Availability: Yeasts require a balanced supply of nutrients, including nitrogen, phosphorus, and micronutrients, for growth and efficient fermentation.
- Sugar Concentration: The sugar concentration should be maintained within a suitable range to prevent inhibition of yeast growth and ethanol production. Excessive sugar concentrations can lead to osmotic stress and reduced fermentation efficiency.
Fermentation can be conducted in batch or continuous processes. In batch fermentation, a defined volume of medium containing sugar and nutrients is inoculated with yeast and allowed to ferment until the desired ethanol concentration is reached.
In continuous fermentation, a steady stream of medium is fed into a fermenter while the fermented broth is continuously removed, allowing for more efficient and consistent ethanol production.
Several challenges can arise during fermentation, including bacterial or fungal contamination and inhibition of yeast activity by high ethanol concentrations, accumulation of byproducts, and nutrient limitation.
4. Uses of Ethanol
Since ancient times, ethanol has been used for a wide range of applications. It is used as a component of alcoholic beverages, a solvent, a raw material for chemical synthesis, and a fuel.
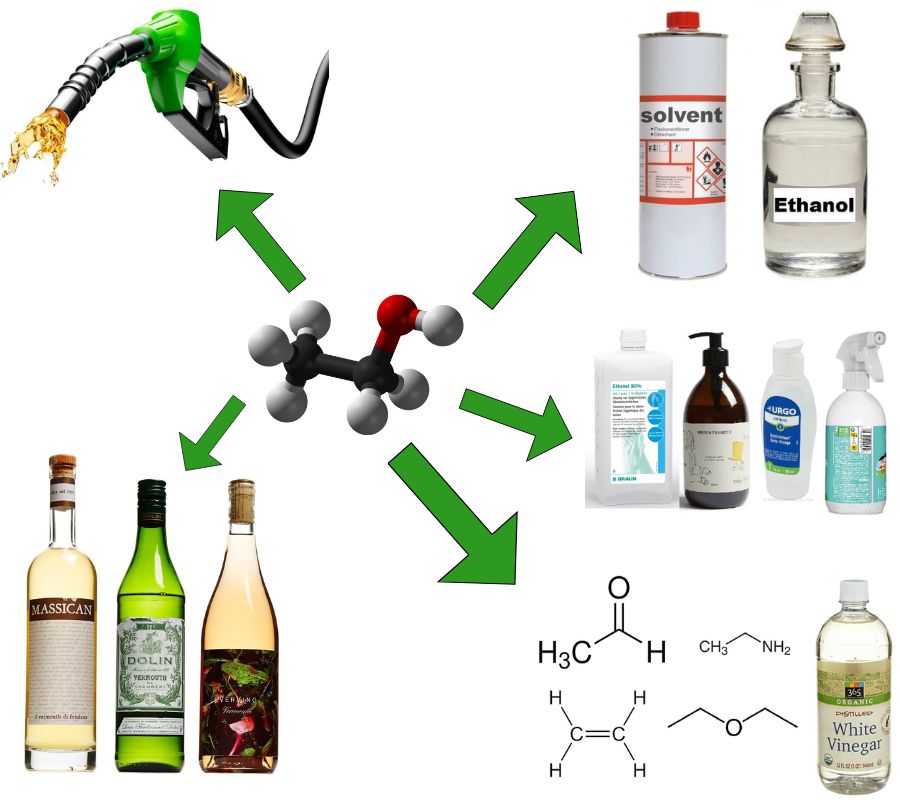
Historically, fermentation-derived ethanol has been primarily used for beverages and specialty chemicals, while chemically synthesized ethanol has been used for industry. However, certain countries, such as Brazil and India, use fermentation ethanol for industrial applications.
To facilitate the use of ethanol in industrial processes at a lower cost, many countries have implemented tax-free policies for industrial and denatured ethanol. These policies often include financial, administrative, and chemical controls to prevent the misuse of tax-free ethanol for beverages.
Based on these regulations, industrial ethanol is categorized into four types: completely denatured alcohol, proprietary solvents and special industrial solvents, specially denatured alcohol, and pure (absolute) ethanol.
Ethanol is frequently added to gasoline as a fuel additive, usually in ratios that range from 5% to 85% ethanol (known as E5 to E85) or as a gasoline extender. Due to its ability to be produced from a number of resources, such as corn, sugarcane, and cellulosic materials, ethanol is becoming more and more popular as a fuel source.
In addition to its use in traditional alcoholic beverages, ethanol is also used in the production of various flavored liqueurs and as a base for many cocktails.
Ethanol is a widely used solvent, second only to water, primarily in the production of toiletries, cosmetics, detergents, disinfectants, pharmaceuticals, surface coatings, and food and drug products.
While both synthetic and fermentation ethanol can be used as solvents, fermentation ethanol is generally preferred, especially in Europe, for applications involving human consumption or body use.
Ethanol is a powerful disinfectant that is commonly used in hospitals, laboratories, and other healthcare settings to kill bacteria, viruses, and other harmful microorganisms. It is also used in the production of hand sanitizers and disinfectant sprays.
Ethanol is used as a raw material for the production of various chemicals, including acetaldehyde, butadiene, diethyl ether, ethyl acetate, ethylamines, ethylene, glycol ethers, and other products formed by reactions with ethylene oxide or epoxides and vinegar.
Some countries, such as Brazil, have established ethanol-based chemical industries for producing chemicals like acetaldehyde that are traditionally derived from petrochemicals in countries with abundant crude oil resources.
Brazil was the world’s largest producer of ethanol from sugar, with over 95% of the total production in 1985–1986. Most of the ethanol produced in Brazil is used as a fuel, while a smaller fraction is employed as a chemical feedstock.
In 1978, India used over 50% of its total ethanol production for the chemical manufacturing of products such as acetaldehyde, acetic acid, acetic anhydride, DDT, organic acetates, acetone, butanol, polyethylene, polyvinyl chloride, and styrene.
5. Toxicology of Ethanol
Ethanol exhibits acute oral toxicity in rats, with LD50 values ranging from 11.5 to 13.7 g/kg. Similar LD50 values of 9.5, 9.6, 9.9, and 6 g/kg have been reported in mice, guinea pigs, rabbits, and dogs, respectively.
Ethanol can cause mild to severe irritation in the rabbit eye, depending on the concentration and amount used. However, it is not significantly irritating to intact skin.
Inhalation of ethanol can lead to irritation of the mucous membranes, excitation, ataxia, drowsiness, narcosis, and ultimately, death due to respiratory failure. Lethal concentrations for mice, guinea pigs, and rats range from 20,000 to 40,000 ppm after several hours of exposure. Concentrations below 6000 ppm can generally be tolerated without intoxication symptoms.
Repeated oral administration of ethanol to juvenile rats at a dose of 10 g/kg/day for 12 weeks resulted in low weight gain and fatty liver degeneration. In monkeys fed a diet containing 40% of total calories as ethanol, disturbances in triglyceride, cholesterol, and phospholipid metabolism were observed, along with fatty degeneration in the liver and myocardium.
Ethanol administration to pregnant rats at levels of 5 mL/kg increased embryolethality and retarded fetal development. However, major malformations were not induced in the fetus.
Ethanol doses of 1–1.5 g/kg caused dominant lethal mutations in male mice but not in female mice. In the Ames test, ethanol did not exhibit mutagenic activity. However, an increase in chromatid breakage was observed in human fibroblast cultures, which is likely a cytotoxic effect rather than a sign of mutagenicity.
Ethanol is primarily metabolized in the liver through three enzyme systems:
- Alcohol dehydrogenase
- Catalase acting as a peroxidase and coupled with a system supplying oxygenated water
- A microsomal ethanol-oxidizing system requiring NADPH as a coenzyme
Approximately 80% of absorbed ethanol is initially metabolized to acetaldehyde, followed by further oxidation to acetic acid. Acetic acid is then degraded to carbon dioxide and water. The oxidation of acetaldehyde to acetic acid is generally faster than the oxidation of ethanol to acetaldehyde.
Ethanol intake in humans can produce various symptoms depending on the concentration, as listed in Table 2. However, individual responses to ethanol can vary significantly.
Symptom | Blood Ethanol Concentration, % |
---|---|
Beginning of Uncertainty | 0.06 - 0.08 |
Slow Comprehension | 0.10 |
Stupor | 0.11 - 0.15 |
Drunkenness | 0.16 |
Severe Intoxication | 0.2 - 0.4 |
Death | 0.4 - 0.5 |
In individuals suffering from alcoholism, fatty liver degeneration is often attributed to increased fatty acid synthesis from acetate, increased lipid transport from peripheral fat depots to the liver, and decreased fatty acid oxidation.
Fatty infiltration of the myocardium and chronic leptomeningitis are also reported in chronic alcoholism and are associated with specific symptoms.
The ACGIH, OSHA, and MAK exposure limits for ethanol are all set at 1000 ppm (1900 mg/m³).
References
- Ethanol; Ullmann’s Encyclopedia of Industrial Chemistry. – https://onlinelibrary.wiley.com/doi/10.1002/14356007.a09_587.pub2
- Ethanol; Kirk‐Othmer Encyclopedia of Chemical Technology. – https://onlinelibrary.wiley.com/doi/10.1002/0471238961.0520080112150719.a01.pub2