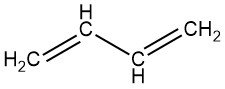
1,3-Butadiene is a colorless gas with a gasoline-like odor. It is the simplest member of the series of conjugated dienes, which contain the structure C=C-C=C, the C being carbon.
Butadiene typically refers to 1,3-butadiene [106-99-0], a highly significant unsaturated C4 hydrocarbon. Its thermodynamically less stable counterpart, 1,2-butadiene [590-19-2], has negligible industrial relevance.
1,3-Butadiene: CH2=CH–CH=CH2
1,2-Butadiene: CH2=C=CH–CH3
Butadiene stands tall as a crucial petrochemical, with global production exceeding 10 million tons annually.
Table of Contents
1. Physical Properties of Butadiene
Butadiene is a colorless gas under normal conditions. Some physical properties are summarized in the following table:
Property | Value |
---|---|
Chemical formula | C4H6 |
Molar mass | 54.09 g/mol |
Boiling point | -4.4 °C |
Melting point | -108.9 °C |
Critical temperature | 425 K |
Critical pressure | 4.32 MPa |
Critical molar volume | 221 cm³/mol |
Density (liquid, 0 °C) | 0.646 g/cm³ |
Density (liquid, 25 °C) | 0.616 g/cm³ |
Density (liquid, 50 °C) | 0.582 g/cm³ |
Gas density (relative to air) | 1.87 |
Vapor pressure (-4.4°C) | 101.3 kPa |
Vapor pressure (0 °C) | 120.0 kPa |
Vapor pressure (25 °C) | 273.6 kPa |
Vapor pressure (50 °C) | 537.9 kPa |
Vapor pressure (75 °C) | 986.7 kPa |
Vapor pressure (100 °C) | 1733 kPa |
Viscosity (liquid, 0 °C) | 0.25 mPa·s |
Viscosity (liquid, 50 °C) | 0.20 mPa·s |
Enthalpy of vaporization (25 °C) | 20.86 kJ/mol |
Enthalpy of vaporization (-4.4 °C) | 22.47 kJ/mol |
Enthalpy of formation (gaseous, 298 K, 101.3 kPa) | 110.0 kJ/mol |
Free enthalpy of formation (gaseous, 298 K, 101.3 kPa) | 151.5 kJ/mol |
Enthalpy of combustion (gaseous, 298 K, 101.3 kPa) | 2541.5 kJ/mol |
Enthalpy of hydrogenation to butane (gaseous, 298 K, 101.3 kPa) | 235.7 kJ/mol |
Entropy of formation (liquid, 298 K, 101.3 kPa) | 199.0 J·mol⁻¹·K⁻¹ |
Enthalpy of melting (164.2 K, 101.3 kPa) | 7.988 kJ/mol |
Butadiene has limited solubility in water. It is soluble in methanol and ethanol, and highly soluble in high-boiling polar solvents like methylpyrrolidone.
Several technical properties are important for safe handling of butadiene. These include:
- Flash Point: -85 °C
- Ignition Temperature: 415 °C
Unstabilized or insufficiently stabilized butadiene can react with atmospheric oxygen to form explosive peroxides.
2. Chemical Properties of Butadiene
Butadiene, possessing two conjugated double bonds, participates in diverse reactions, including 1,2- and 1,4-additions with itself (polymerization) and other reagents, linear dimerization, trimerization, and ring formation.
Polymerization
The most significant reaction for butadiene involves polymerization through 1,2- and 1,4-addition, leading to a variety of polymers. The nature of these polymers depends on preparation methods and the catalyst system employed, resulting in industrially important synthetic rubbers and polymer resins.
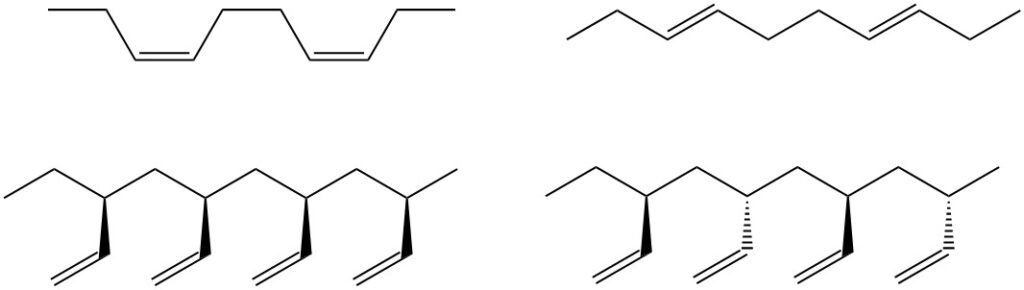
Other Addition Reactions
Butadiene undergoes 1,2- or 1,4-addition with various reagents, with the proportions of addition products depending on reaction conditions. These addition products serve as crucial intermediates in the production of substances such as chloroprene and hexamethylenediamine.
Specific Reactions
1. Chlorination (Chloroprene Production): In a typical example of electrophilic addition, butadiene reacts with chlorine to yield chloroprene (1), a compound with multiple industrial applications.
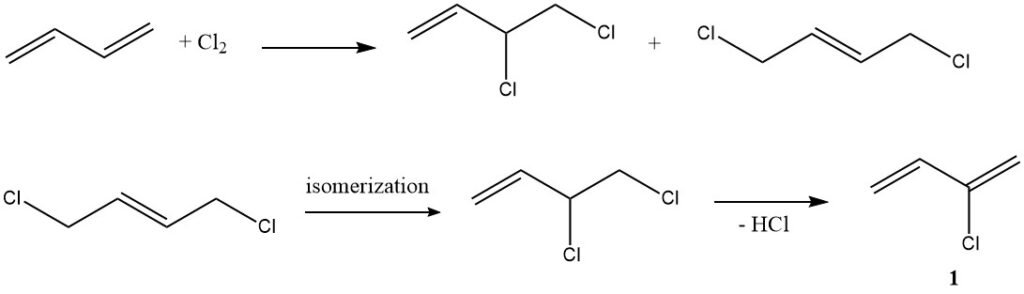
2. Hydrocyanation (Adiponitrile Production): Employing a three-step process developed by Du Pont, butadiene undergoes hydrocyanation to form a mixture of isomeric linear and branched pentene nitriles. Subsequent isomerization and further hydrocyanation result in adiponitrile (2).
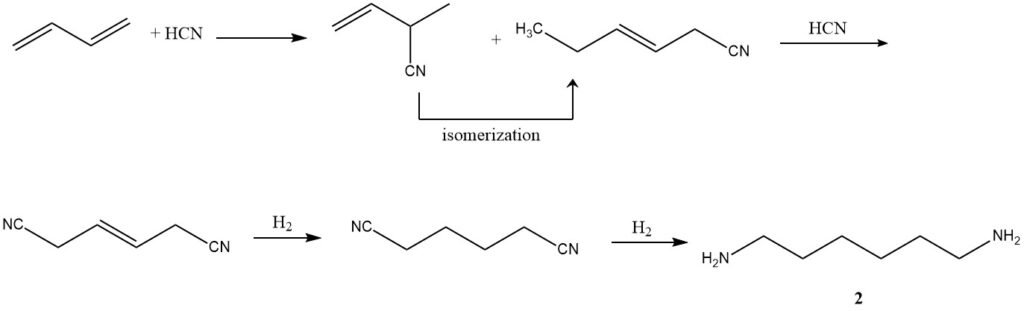
3. Carboalkoxylation (ε-Caprolactam Production): DSM and Du Pont have explored an alternative process for ε-caprolactam production from butadiene. This multi-step process involves carboalkoxylation, hydroformylation, and reductive amination. Although not yet implemented on an industrial scale, it presents a promising avenue for future development.
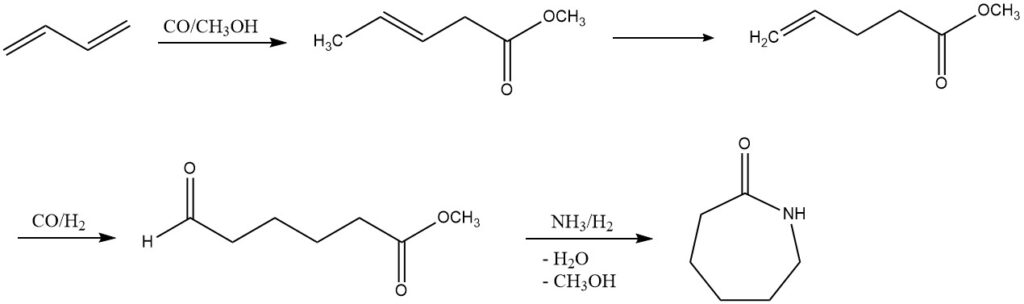
4. Acetoxylation (1,4-Butanediol or Tetrahydrofuran Production): Through acetoxylation, butadiene leads to the synthesis of 1,4-diacetoxy-2-butene, which, in a multi-step Mitsubishi process, is converted into 1,4-butanediol or tetrahydrofuran. These processes, under the influence of specific catalysts, showcase the potential for diverse chemical transformations.
5. Other Oxidation Reactions: Innovative processes, such as the Toyo Soda process and direct oxidation with air or oxygen, represent economic methods for the production of various C4 derivatives. The introduction of 3,4-epoxy-1-butene through oxidation opens avenues for the creation of valuable chemical intermediates.
6. Diels-Alder Reaction: One of the most well-known reactions of butadiene, the Diels-Alder reaction, plays a crucial role in industrial processes for the production of styrene. Dimerization of butadiene and subsequent conversion to styrene highlights the versatility of this reaction in synthesizing valuable chemical compounds.

7. Cyclodimerization, Cyclotrimerization: Two molecules of butadiene can undergo cyclodimerization to produce 1,5-cyclooctadiene, while three molecules result in 1,5,9-cyclododecatriene. These cyclic compounds, facilitated by specific catalysts, serve as intermediates in the production of higher polyamides.
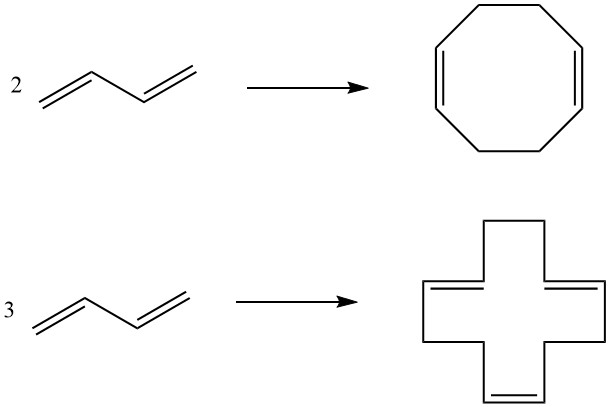
8. 1,4-Cycloaddition: Reversible 1,4-addition of butadiene with sulfur dioxide leads to the formation of the cyclic sulfone 2,5-dihydrothiophene-1,1-dioxide, further converted into sulfolane. This process showcases the adaptability of butadiene in the synthesis of highly polar solvents.

9. Linear Dimerization and Trimerization; Telomerization: Under the influence of Ni, Co, Pd, or Fe catalysts, butadiene undergoes linear dimerization or trimerization. The resulting linear oligomers, separated by chain length, offer diverse compounds, such as 1,3,7-octatriene.
Telomerization reaction is the di- and oligomerization of a 1,3-diene under addition of a nucleophilic compound. It is catalyzed by Pd, Ni, or Pt and yields a broad spectrum of products depending on the nucleophile used.
10. Hydrogenation: Selective hydrogenation of butadiene generates 1-butene or 2-butene, and careful adjustment of reaction conditions ensures sufficient conversion without over-hydrogenation to n-butane. Commercial processes from companies like Axens, UOP, and Lummus provide tailored approaches to maximize the yield of specific butene isomers.
11. Formation of Complexes: Butadiene forms complexes with various metal compounds, such as Cu(I) salts. While historically used in the extraction of butadiene, modern extractive distillation techniques have largely replaced this method. Complexes with other transition metals like Fe, Ni, Co, Pd, and Pt are well-established, contributing to mechanistic studies in alpha-olefin polymerization catalysis.
3. Production of Butadiene
Historically, diverse methods existed for butadiene production. Today, four main pathways remain:
- Naphtha Cracking: This dominant route captures butadiene as a byproduct of ethylene production.
- Butane/Butene Dehydrogenation: This process plays a minor, campaign-based role depending on feedstock and butadiene prices.
- Ethanol Production: This method has diminished in importance.
- Acetylene Production: This route is no longer commercially viable.
Emerging bio-based approaches are under exploration.
The economics of butadiene production are heavily influenced by the coupled production of ethylene and propylene. When these C2 and C3 olefins are inexpensive, dedicated butadiene production from butenes and butane becomes more attractive.
3.1. Production From Acetylene
Historically, two pathways were employed to produce butadiene from acetylene. Both are now obsolete due to the energy-intensive and expensive nature of acetylene production. Only the initial steps of the Reppe process, leading to 1,4-butanediol and tetrahydrofuran, remain commercially relevant.
3.2. Production From Ethanol
The IPATJEW and OSTROMISLENSKY process, subsequently modified by LEBEDEW, once served as a key source of butadiene, especially in Germany and Eastern Europe during World War II. This process is illustrated by the reaction:
2 CH3CH2OH → CH2=CHCH=CH2 + 2 H2O + H2
and operated at temperatures ranging from 370 to 450 °C using MgO – SiO2 or SiO2 – Al2O3 catalysts, it achieves selectivities of up to 70 %.
While no longer competitive for large-scale production, it may still be viable in regions with cheap ethanol or surplus agricultural alcohol.
3.3. Dehydrogenation of Butane and Butenes
The dehydrogenation of butane and butenes to produce butadiene presents unique challenges due to its endothermic nature. To maximize yield and selectivity, several key considerations must be addressed:
Le Chatelier’s Principle: Lowering the partial pressure of the desired products, butadiene and hydrogen, drives the reaction forward. This can be achieved through vacuum operation or by adding steam to the reaction mixture.
Benefits of Steam:
- Steam helps mitigate catalyst deactivation by preventing coke formation on the catalyst surface.
- The endothermic nature of the dehydrogenation reaction requires external heat input. Steam readily condenses, releasing significant heat to drive the reaction.
- The condensed steam can be easily separated from the product stream, ensuring minimal contamination.
While higher temperatures favor butadiene formation, they also increase the risk of undesirable side reactions such as cracking, isomerization, and polymerization. Finding the optimal temperature balance between yield and selectivity is crucial.
Compounds susceptible to the aforementioned side reactions are removed before the dehydrogenation process to minimize their influence.
As the dehydrogenation reaction does not proceed to completion, the product stream requires separation to isolate butadiene. Unreacted starting materials are recycled back into the process to maximize efficiency.
By carefully controlling these aspects, the dehydrogenation of butane and butenes can be optimized for efficient and selective butadiene production.
3.3.1. Dehydrogenation of n-Butane
Two prominent methods exist for dehydrogenating n-butane to butadiene:
1. Houdry-Catadiene Process:
This established one-step process operates commercially since 1943. Multiple packed-bed reactors, arranged parallel, are cycled between reaction and regeneration phases. An aluminum oxide catalyst containing 20% chromium oxide facilitates the dehydrogenation of pure n-butane or its mixture with n-butenes at 550-650 °C and 10-25 kPa.
High temperatures favor butadiene production but also generate undesirable byproducts like C1-C3 hydrocarbons, hydrogen, and catalyst-fouling coke.
After each reaction cycle (5-15 minutes), the reactor undergoes regeneration: burning the coke deposit releases heat, stored in the catalyst and inert material, for the next reaction phase.
This process yields a 15-18% butadiene concentration at the reactor outlet. Subsequent recovery steps, including quenching, compression, stripping, and separation, increase butadiene concentration to 30-50%. From 1000 tons of n-butane, roughly 550 tons of butadiene are obtained. Pure butadiene can be isolated by extractive distillation.
2. Phillips Petroleum Process:
This two-step process offers advantages like longer catalyst life and higher butadiene yields.
- Dehydrogenation to Butene: n-Butane undergoes catalytic dehydrogenation to butene at 600°C and 1 bar on a Cr2O3-Na2O-Al2O3 catalyst.
- Butene Separation: n-Butenes are extracted using auxiliaries like acetone, acetonitrile, or furfural.
- Butadiene Formation: Separated n-butenes are dehydrogenated to butadiene in an isothermal tubular reactor. The reactor is heated by flue gas to 600 °C and operates at 1 bar pressure with superheated steam addition over a Fe2O3-K2O-Al2O3 catalyst.
- Butadiene Recovery and Purification: Extractive distillation with the aforementioned auxiliaries recovers and purifies butadiene.
The Phillips process boasts longer running times (no catalyst regeneration) and a 65 % butadiene yield based on n-butane, demonstrating its efficiency and improved selectivity compared to the Houdry-Catadiene process.
3.3.2. Dehydrogenation of n-Butenes
n-Butenes, commonly found in cracked gasoline, olefin production, and LPG dehydrogenation, are often present as part of a C4 hydrocarbon mixture. While C4 paraffins can be separated from C4 olefins via extractive distillation (as in butadiene recovery), separating isobutene and n-butene poses a unique challenge due to their close boiling points.
Several methods address this separation hurdle:
1. BASF Process: Utilizes 40-45% H2SO4 to selectively convert isobutene to tert-butanol, allowing easier separation.
2. Isobutene can be converted to methyl tert-butyl ether or isobutene oligomers for further processing and separation.
3. Distillation with Hydroisomerization: Processes like Axens’ Isopure and CD-Tech’s CD DeIB combine distillation with hydroisomerization of 1-butene to 2-butene. Since 2-butene exhibits a distinct boiling point from isobutene, distillation becomes feasible.
4. Dow Process: Employs dehydrogenation of n-butenes with steam over a Ca/Ni phosphate catalyst stabilized with Cr2O3. Superheated steam provides the necessary heat for the reaction, achieving 50% butene conversion and up to 90% butadiene selectivity.
5. Parallel Reactor Approach: As the Dow process requires catalyst regeneration after 15 minutes, parallel reactors are employed, enabling continuous operation.
Similar processes have also been developed by Shell and Phillips Petroleum, showcasing the diverse approaches available for n-butene dehydrogenation and butadiene production.
3.3.3. Oxidative Dehydrogenation of n-Butenes
Oxidative dehydrogenation (ODH) offers several advantages over conventional dehydrogenation processes for butadiene production. By removing hydrogen from the equilibrium through oxidation, ODH significantly improves conversion and selectivity:
2 C4H8 + O2 → 2 C4H6 + 2 H2O
This exothermic reaction provides heat for the endothermic dehydrogenation, further reducing energy requirements. Additionally, the presence of oxygen and steam helps minimize catalyst coking.
Historical ODH Processes:
- Oxo-D Process (Petro-Tex): This process, employed since 1965, utilizes a ferrite catalyst and operates under mild pressure. The advantages include low steam and heating energy consumption, high conversion/selectivity, long catalyst life, and no regeneration requirement.
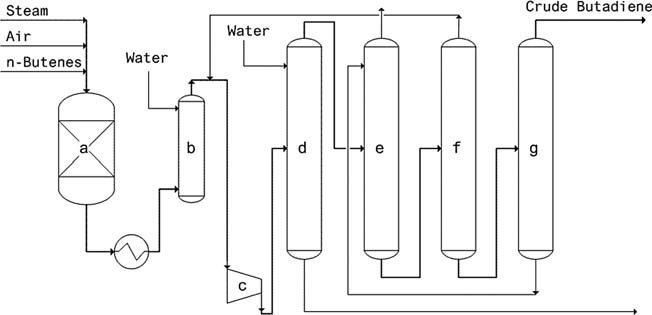
a) Reactor, b) Quench tower, c) Compressor, d) Scrubber, e) Absorber, f) Degasser, g) Stripper
- O-X-D Process (Phillips): Operating since 1976, this process employs a Li-Sn-P-O catalyst and achieves 75-80% butene conversion with 88-92% butadiene selectivity.
Newer Developments in ODH:
- Nippon-Zeon Process: This process uses mixed n-butene/butane and air/off-gas as feed, offering flexibility in feed composition. A mixed metal oxide catalyst achieves 85% butadiene yield based on the butenes.
- Mitsubishi Chemical Process: This process utilizes a Mo/Bi/Co catalyst and converts a C4 mixture containing 70% n-butene with air, steam, and nitrogen. The addition of nitrogen allows for a high oxygen/butane ratio, minimizing coking. Butadiene conversion exceeds 80% with a selectivity above 90%.
- Other Developments: Asahi Kasai and Mitsui Chemicals have also developed ODH processes with promising results, including fluidized bed reactors and ethylene-to-butadiene conversion strategies.
Overall Benefits of ODH:
- Higher conversion and selectivity compared to conventional dehydrogenation processes.
- Reduced energy requirements due to the exothermic oxidation reaction.
- Lower catalyst coking.
- Potential for using mixed C4 streams as feedstock.
Limitations:
- ODH processes are generally more complex than conventional dehydrogenation.
- The high temperatures required can lead to undesired byproduct formation if oxygen is used with butane
3.4. Isolation of Butadiene from C4 Steam Cracker Fractions
Butadiene is isolated primarily from C4 fractions generated during the steam cracking of hydrocarbons. The choice of feedstock, such as naphtha in Western Europe and LPG in the United States, plays a significant role in the process, while cracking severity primarily influences the C4 fraction composition.
Separating butadiene from this mixture presents a challenge due to its azeotropic behavior with butane. Consequently, specialized techniques are necessary.
The cuprous ammonium acetate process, utilizing its ability to form a complex with butadiene, tackles this issue for fractions with low butadiene and C4 acetylene content. However, higher levels of acetylenes require pre-treatment via selective hydrogenation.
Extractive distillation emerges as the preferred method for butadiene isolation. This technique exploits the varying affinities of different components towards specific organic solvents based on their degree of unsaturation.
Popular solvents include acetonitrile ( by Shell/KBR and LyondellBasell), N-methylpyrrolidone (by BASF), dimethylformamide (by Nippon Zeon Chemicals), and furfural (by ConocoPhillips).
These processes, involving one or two extractive distillation steps coupled with conventional distillation, separate butadiene and other high-affinity components from less-soluble butanes and butenes. Further purification steps remove remaining impurities to achieve butadiene purity.
The BASF process, employing NMP as a solvent, boasts noncorrosiveness and high butadiene yields, while the Nippon Zeon DMF process exhibits similar advantages. Recent advancements in this field include dividing wall technology, combining two distillation steps in one unit to enhance efficiency, and improved distillation internals and process control to boost existing units’ capacity and performance.
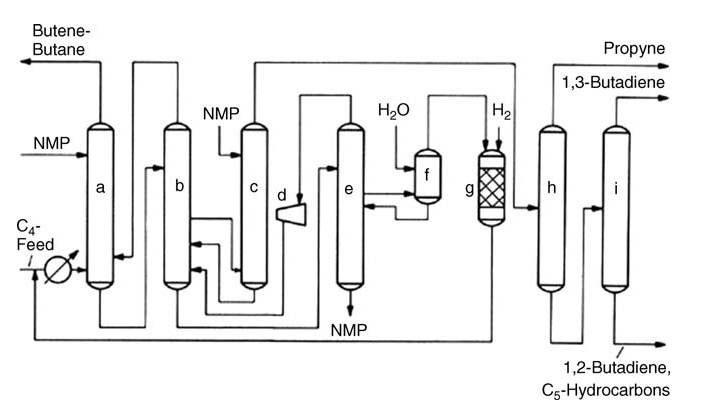
a) Main washer; b) Rectifier; c) After washer; d) Centrifugal compressor; e) Degassing tower; f) Water washer; g) Hydrogenation reactor; h) First distillation; i) Second distillation
Alternative approaches like selective hydrogenation of C4 acetylenes offer various benefits. UOP’s KLP process removes acetylenes before extraction, simplifying the process and increasing butadiene yield. CDTECH’s CDHYDRO process provides flexibility by allowing varying levels of acetylene conversion, minimizing butadiene loss.
3.5. Butadiene from Bio-Based Resources
The growing trend of utilizing renewable resources for petrochemical production extends to butadiene. While various pathways exist, routes using bioalcohols hold the most promise.
Bioethanol production, established through fermenting sugarcane, corn, or lignocellulosic material, reached 68 million tons globally in 2011. Existing technologies can convert bioethanol into butadiene.
Although no large-scale bio-butadiene production exists currently, biobutanol’s increasing interest as a fuel additive fuels ongoing process development. Dehydration and dehydrogenation of biobutanol to n-butene.
Another potential route involves gas fermentation of carbon monoxide, obtained from organic material gasification or industrial waste gases. This bacterial process produces 2,3-butanediol, a precursor to butadiene. However, this technology is still in its early stages.
Currently, bio-based butadiene production lacks economic competitiveness compared to the petrochemical route. However, rising oil and natural gas prices, coupled with further technological advancements, could favor bio-transformation routes in the future.
4. Uses and Economic Importance of Butadiene
Butadiene is primary used as a monomer or comonomer in synthetic rubber production. It contributes to styrene-butadiene rubber, polybutadiene rubber, acrylonitrile-butadiene rubber, and chloroprene rubber.
Beyond rubber production, butadiene plays a crucial role in several plastics. It forms the backbone of impact-resistant polystyrene, a two-phase system combining polystyrene and polybutadiene. Additionally, butadiene contributes to ABS polymers, a blend of acrylonitrile, butadiene, and styrene, and MBS copolymers, used as modifiers for polyvinyl chloride.
Furthermore, butadiene serves as an intermediate in various chemical syntheses.
In the United States, Japan, and Western Europe, the automobile industry constitutes the primary consumer of butadiene rubber.
Butadiene prices experienced a significant drop in the early 1990s due to oversupply from increased cracking capacities. This led to butadiene hydrogenation and use as cracker feedstock.
Since the late 1990s, surging demand for rubber products and polymers, particularly in Asia, fueled an exponential increase in butadiene demand and a steady price rise.
The global economic recession of 2008 triggered a dramatic drop in butadiene demand, followed by a recovery. Butadiene prices surpassed ethylene prices, a rare occurrence, until volatility led to peaks exceeding 2,000 euros per ton.
These price fluctuations can be attributed to the arrival of ethane-based crackers in the Middle East and the shift of US crackers to lighter gas feedstock. These changes result in a reduced butadiene yield despite increasing global demand.
5. Toxicology of Butadiene
Exposure and Toxicity:
- Occupational exposure to butadiene is highest in petrochemical, butadiene purification, and processing industries, and in the manufacture of rubber and plastic products.
- Butadiene primarily enters the body through inhalation, with skin contact a less frequent occurrence.
- Non-occupational exposure can occur near point sources like fires, cigarette smoke, and vehicle emissions, though at much lower levels than in occupational settings.
Animal Studies:
- Butadiene exhibits low acute toxicity, with high doses causing narcosis preceded by excitation and hyperventilation.
- Repeated short-term exposure at high concentrations (4000-8000 ppm) causes salivation in rats but does not affect growth, food consumption, or other parameters.
- Mice exposed to similar levels show decreased body weight and increased mortality, with higher sensitivity to butadiene compared to rats.
- Long-term exposure in rats (up to 111 weeks) leads to transient nasal secretion, ataxia, and early mortality at the highest concentration (8000 ppm). It also increases liver weight and suggests a potential for metaplasia in the lung and higher incidence of some tumors.
- Mice exposed to lower concentrations (625-1250 ppm) for 60-61 weeks exhibit toxic and proliferative lesions in the nasal cavity, liver necrosis, and atrophy of reproductive organs. Notably, they develop various tumors, including malignancies, earlier and at higher rates than controls.
- A two-year study with even lower concentrations (6.25-625 ppm) reveals the early development of extensive lymphomas, unusual heart tumors, and lung tumors in mice.
Metabolism and Mutagenicity:
- Butadiene is metabolized in the liver to form epoxide intermediates, which are suspected to be the key contributors to its toxicity and carcinogenicity.
- The formation of these metabolites is more pronounced in mice than in rats and humans.
- Butadiene exhibits mutagenicity in the Ames test only in the presence of enzymes, suggesting the formation of a mutagenic intermediate.
Human Data:
- Limited human data is available on the effects of butadiene exposure.
- Volunteers exposed to a very high concentration (8000 ppm) experienced temporary irritation, blurring vision, and respiratory symptoms, but no cumulative effects were observed.
- Hematology studies of workers exposed to average levels of 20 ppm butadiene showed no significant abnormalities.
- Early mortality studies of workers in the styrene-butadiene rubber industry did not suggest an increased risk of cancer.
- Recent reviews of epidemiological studies provide evidence for an increased risk of hematolymphatic malignancies, particularly leukemia, with elevated butadiene exposure.
Classification and Workplace Exposure Limits:
- Based on animal and human data, butadiene is classified as a Group 1 carcinogen by the International Agency for Research on Cancer (IARC).
- Occupational exposure limits vary slightly between different regulatory bodies, with limits ranging from 0.5 to 4.4 mg/m3.
- Continuous efforts are being made to reduce exposure levels in workplaces handling butadiene.
Reference
- Butadiene; Ullmann’s Encyclopedia of Industrial Chemistry. – https://onlinelibrary.wiley.com/doi/10.1002/14356007.a04_431.pub2